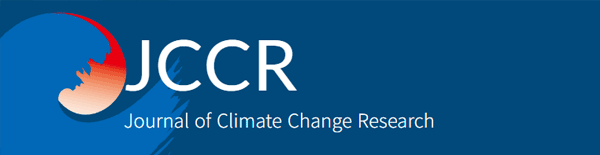
대기 중 온도 및 CO2 농도 처리에 따른 구상나무, 소나무, 졸참나무 유묘의 생장 및 생리적 반응 특성
Abstract
This study was conducted to investigate the effects of elevated air temperature and atmospheric CO2 concentration on the growth and physiological characteristics of two‐year‐old seedlings of three representative species (Abies koreana, Pinus densiflora, and Quercus serrata) in Korea based on a climate change scenario. Our study used a temperature gradient chamber (TGC; aC‐eT) and CO2‐temperature gradient chamber (CTGC; eC‐eT) to simulate climate change conditions under ambient and elevated CO2 concentration and air temperature. Net photosynthetic rate (Pn) and biomass were measured and carboxylation efficiency (CE) and biomass allocation were calculated to examine the physiological responses of the seedlings. A. koreana and P. densiflora were negatively impacted by warming but Pn and CE increased under the eC‐eT condition while Q. serrata showed decreased Pn and CE during the second year. There were significant differences in total biomass in P. densiflora, but showed no differences in Q. serrata. Shoot/root ratio in Q. serrata showed a significant increase under the aC‐eT condition. Our results highlight that further investigation of species‐specific physiological responses and carbon allocation under artificial climate conditions is needed.
Keywords:
Climate Change, Physiology, Net photosynthetic rate, Biomass allocation1. 서 론
유엔 기후변화 정부간 패널 (IPCC)의 지구온난화 특별보고서 (IPCC, 2018)에 따르면, 1970년 이후로 지구의 평균기온이 한 세기에 1.7°C씩 증가하고 있으며 (Marcott et al., 2013), 대기 중 CO2 농도는 지난 2000년부터 10년에 20 ppm씩 증가하면서, 과거 80만년 동안 증가한 평균에 비하여 10배 가까이 빠른 속도로 증가하고 있다고 한다 (Lüthi et al., 2008; Bereiter et al., 2015). 대기 중 온도와 CO2 농도 상승은 식물의 광합성과 생장에 직·간접적으로 영향을 주어 식물 분포역의 변화, 식물의 생장량 변화 등 다양한 결과로 이어 질 수 있다 (Woodward, 1987). 기후변화로 인해 대기 중 온도와 CO2 농도가 증가하게 되면, 식물체 내 CO2 분압이 높아져 대기에서 광합성기관으로의 CO2 확산이 촉진되고 rubisco의 탄소고정반응을 촉진 시켜 그 결과 광합성 속도를 높임으로써 식물체의 생장이 촉진된다고 보고된 바 있다 (Stirling et al., 1998; Kim and Lee, 2001). 그러나 선행 연구에 따르면 수종에 따라서 장기간 고농도 CO2 노출에도 광합성 속도와 능력에 대한 반응이 작게 나타나거나 (Sage et al., 1989; Sendall et al., 2015), 오히려 감소하기도 한다 (Medlyn et al., 1999; Ainsworth and Rogers, 2007, Norby et al., 2010). 또한 CO2 시비효과에 의한 광합성 활성과 지상부·지하부 생물량 증가는 식물의 양분, 수분, 온도 등 다른 환경요인 변화에도 영향을 받으므로 기후변화로 인한 수목의 반응은 복잡하고 다양하다 (Oren et al., 2001; Huang et al., 2007). 일반적으로 대기 중 CO2 농도의 증가는 잎의 광합성 속도를 증가시킬 것으로 예측되고 있지만 CO2 시비효과로 인한 광합성 촉진에 영향하는 다른 환경요인을 배제하기 어려워 실제 효과에 대해서는 분명하게 밝혀지지 않았다 (Leakey et al., 2009; Zhu et al., 2017). 이러한 점으로 보아 제한적인 조건하에서 대기 중 온도와 CO2 농도 변화에 따른 수목의 반응을 정량적으로 나타내기 위한 연구가 필요하다.
우리나라의 산림생태계에서도 기후변화 영향이 나타나고 있는데 특히 한라산, 지리산 일대에서 집단으로 쇠퇴하는 현상을 보이고 있는 구상나무의 경우, 아고산 일대에 제한적으로 분포하고 있는 자생수종으로 최근 겨울철 고온과 극심한 가뭄 등의 이상기상이 겹쳐 발생함에 따라 멸종위기에 당면하고 있다 (Koo et al., 2001; Lim et al., 2006; Shin and Lee, 2008). 우리나라 면적 대비 가장 많은 비율을 차지하고 있는 소나무의 경우에도 고온과 가뭄으로 인한 피해 사례가 발생하고 있으며, 기후변화시나리오 (RCP) 4.5와 8.5에 따라 우리나라 소나무의 적지분포 경계가 동쪽과 북쪽으로 이동하고 (Chun and Lee, 2013), 참나무류 수종 중 상수리나무와 신갈나무 적지분포가 일부 지역에 제한적으로 분포할 것으로 전망되며 (KFRI, 2014b) 이들 수종의 기후 및 환경변화와 밀접한 연관이 있는 연륜생장 또한 감소할 것으로 예측되고 있다 (Lim et al., 2006).
따라서 본 연구에서는 기후변화에 민감하게 반응을 보이며, 분포역의 변화가 예상되고 있는 구상나무, 소나무, 졸참나무를 대상으로 대기 중 온도와 CO2 농도가 상승한 미래 대기 환경에 반응하는 광합성적 특성과 생물량 변화를 측정·분석하였다. 이를 통해 3년간의 온도·CO2 처리가 각 수종의 생장과 생리적 특성에 미친 영향을 정량화하고자 한다.
2. 재료 및 방법
2.1 기후환경변화시뮬레이션 시스템 (Temperature Gradient Chamber & CO2·Temperature Gradient Chamber)
온도와 CO2 농도 제어는 경기도 포천시 소홀읍 직동리 51에 위치한 국립산림과학원 산림기술경영연구소의 온도·CO2 구배 시스템온실을 이용하였다. 온도·CO2 구배 시스템온실은 자연 대기 온도 및 CO2 농도의 일변화, 계절변화, 광조건 등을 직접적으로 반영하여 미래 기후변화 환경을 조성할 수 있는 시설이다. 총 4동으로 구성되어있는 각 온실은 3.5 m × 3.0 m × 30.0 m 이며, 온도 구배 온실이 2동 (Temperature Gradient Chamber - TGC), 온도 및 CO2 농도 구배 온실이 2동 (CO2·Temperature Gradient Chamber - CTGC)이다. 온실 입구의 반대쪽에 설치한 환풍기를 가동하여 입구로부터 환풍기가 설치된 출구로 인위적인 공기 흐름이 발생되게 함으로써 온실 내 온도와 CO2 농도의 구배 환경을 조성하였다. 맑은 날에는 외부 대기가 유입되어 온실 내를 이동하면서 일사에 의해 기온이 상승해 출구에 가까워질수록 미래 기후변화 조건으로 환경이 조성된다. 일사량 증감과 기온 변화에 따라 환풍기의 회전속도를 조절함으로써 온도 구배 온실에서는 입구와 출구의 기온차가 5°C 구배를 유지하고, 온도·CO2 구배 온실에서는 기온뿐만 아니라 CO2 농도도 차이 날 수 있도록 CO2 가스 모니터링 및 제어 시스템을 통해 조절하였다 (Lee et al., 2001; Seo et al., 2016). 출구에서는 일정한 온도 상승 조건이 안정적으로 유지되고 있음을 확인하였다 (Table 1).
처리 온도와 CO2 농도는 대조구 (aC-aT)와 RCP 8.5 시나리오를 통해 온실가스 배출 추세를 유지 할 경우, 21세기 후반 (2071∼2100)에 도래할 것으로 예상되는 값으로 설정하였다 (IPCC, 2013). 현재 수준의 대기 중 CO2 농도에 현재 기온보다 5°C 상승된 조건 (aC-eT, ambient CO2 concentration and elevated air temperature)과 대기 CO2 농도의 2.2배에 기온을 현재보다 5°C 증가시킨 환경으로 (eC-eT, elevated CO2 concentration and elevated air temperature) 처리하였다. 온도와 CO2 처리는 2016년 4월부터 2018년 8월까지 실시하였다. CO2 폭로는 매년 매월 4월부터 11월까지 총 8개월간 실시하였으며, 폭로 시간은 오전 7시부터 오후 6시까지로 설정하였다.
2.2 공시재료와 관수 처리
공시재료는 모두 2년생 어린나무로, 지리산 반야봉에서 종자를 채집 후 금원산 거창 휴양림에서 생장한 구상나무 (Abies koreana) 2-0묘 125본과 산림생산기술연구소 양묘장에서 2015년 3월 파종 후, 야외에서 월동한 소나무 (Pinus densiflora)와 졸참나무 (Quercus serrata) 실생묘 각각 125본을 선정하여 온도 및 CO2 처리별로 각 5본씩 배치하였다. 생장이 시작되는 4월부터 11월까지 모든 온실과 처리에서 동일하게 2회/ 1일 관수를 실시하였다. 고온 및 햇빛에 의한 관수 피해를 줄이기 위해 저녁 8시, 새벽 6시에 관수를 실시하였다. 단, 2018년 4월과 5월에는 시설 문제로 모든 온실 내 관수가 이루어지지 않았다.
2.3 광합성 특성 측정
광합성은 전광에 노출된 양엽을 대상으로 측정하였으며, 수종별 순광합성율 (Net photosynthesis rate; Pn)은 LI-6400 휴대용 광합성 측정기 (LI-COR Inc., USA)를 이용하여 측정하였다. 생육이 활발한 기간 중 1회, 처리구별 3회 반복 측정하였다. 측정은 오전 9시에 시작하여 정상적인 측정이 가능한 시간까지 지속하였다. 측정 시 상대습도는 55∼60%로 유지하였고, 챔버로 유입되는 공기유량은 500 μmols-1으로, 챔버 내 기온은 처리구에 따라 aC-aT는 대기온도 (27°C), aC-eT와 eC-eT 처리구는 대기온도+5°C (32°C)로 설정하였다. 각 조건을 설정 후 안정화 시킨 다음 측정을 시작하였다. 침엽수인 구상나무와 소나무의 경우 순광합성율 (μmolm-2s-1)을 측정한 잎을 채취하여 산출한 건중량 (60°C 건조기에 72시간 이상 건조 후 측정)을 이용하여 단위 무게 당 순광합성율로 계산하였고 (μmolg-1s-1), 활엽수인 졸참나무는 엽면적 당 순광합성율로 계산하였다 (μmolm-2s-1). 일반적으로 광합성에서 rubisco 활성과 탄소고정시스템의 능력을 평가하는데 사용되는 탄소고정효율 (Carboxylation Efficiency)은 엽육 내 CO2 농도 (Ci) 변화에 대한 광합성 증가가 직선적으로 이루어지는 0∼100 μmolCO2mmol-1영역에서, 회귀직선 (y=a+bx)의 기울기 b를 이용하여 산출하였다 (Farquhar et al., 1980). 2016년부터 모든 온실에서 처리가 시작되었으며 2018년 구상나무와 소나무는 봄철 관수시설 관리 문제로 대량 고사하였으므로, 광합성 측정이 불가능하였다.
2.4 생물량 분석
광합성 측정을 마친 후 실험목을 수확하여 잎, 줄기, 뿌리로 각각 구분하였고, 그 이후 실험실에서 60°C 건조기에 72시간 이상 건조하여 건중량을 측정하였다. 이를 이용하여 총 물질 생물량과 부위별 배분과 지상부/지하부 (T/R)을 구하였다. 구상나무는 고사로 인한 시료 손실이 많아 생물량 측정자료를 활용한 분석에서 제외하였다.
2.5 통계분석
수종별 고온 및 고농도 CO2에 의한 차이를 확인하기 위해 평균 차이 분석은 분산분석 (ANOVA)을 실시하였다. 이를 통해 유의한 차이가 나타날 경우 (p < 0.05), 처리구 간 비교는 Duncan을 이용하여 사후분석 하였다. 모든 통계 분석은 R 통계 프로그램 (ver. 3.3.2, R Core Team, 2016)을 이용하였다.
3. 결과 및 고찰
3.1 고사율
2018년 봄, 관수 시스템 고장으로 인한 20일 무관수 처리 효과로 3년차 (당시 4년생) 온도·CO2 처리 중인 소나무와 구상나무가 각각 92.0%, 38.0% 고사하였다. 이러한 고사피해는 심한 건조 스트레스 하에서 기공을 닫고 수분손실을 줄이기 위해 엽육 내 CO2와 수분 교환을 억제함으로써 광합성 기능이 저하된 것으로 판단된다 (KFRI, 2014a). 생존한 62.0%의 구상나무도 생육상태가 좋지 않고, 엽량이 적었으므로 소나무와 구상나무의 처리 3년차 광합성 측정이 불가능하였다. 동일한 시기에 졸참나무는 개엽 전이었으므로 고사하지 않았다.
3.2 고온처리에 따른 생리적 반응 특성
고온 조건에 따른 수종별 연도별 순광합성율 반응은 Figure 1과 같다. 생육기간 내 측정한 세 수종 모두 고온 처리에서 순광합성율이 감소하는 경향을 보였으나 통계적인 차이는 없었다. 연도별 온도 증가에 따른 수종별 순광합성율은 구상나무의 경우 2016년, 2017년 각각 대조구보다 약 55.4%, 19.6% 감소하는 경향을 보였다 (Figure 1a). 소나무도 구상나무와 같은 경향으로 2016년과 2017년 각각 약 50.6%, 35.0 % 감소하는 경향을 보였다 (Figure 1b). 졸참나무의 순광합성율은 지속적인 고온 처리에 의해 2년차인 2017년부터 급격히 감소하는 경향을 보이기 시작했으며, 각 연도별 감소율은 9.6%, 51.5%, 43.0%였다 (Figure 1c). Sendall et al. (2015)에 따르면 대기 중 온도 변화에 광합성 기작이 적응하는 능력은 수종마다 차이를 보인다고 한다.
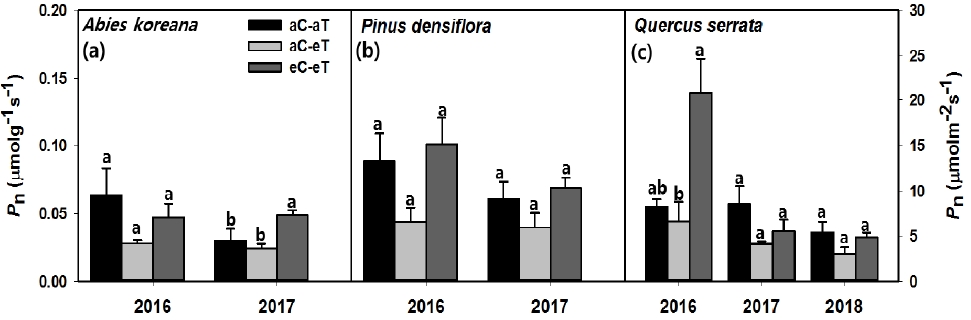
Net photosynthetic rate (Pn) in (a) A. koreana, (b) P. densiflora and (c) Q. serrata grown in ambient (aC-aT), ambient CO2 & warmed treatment (aC-eT), elevated CO2 & warmed treatment (eC-eT) in 2016, 2017 and 2018. Values are the means ± SE (n=3 for each treatment). Different letters within a subpanel represent statistical differences (p <.05) based on the Duncan test. Units were differed as each calculation methodology; ‘μmolg-1s-1’ for A.koreana and P.densiflora and ‘μmolm-2s-1’ for Q.serrata. Error bar indicatstandard error.
Figure 2는 온도 증가가 광합성 능력인 탄소고정효율에 미친 영향을 보여 주며, 대조 환경과 처리구간 효과를 비교하기 위해 처리구/대조구 × 100 (%)으로 나타냈다. 탄소고정효율은 수종 및 폭로기간에 따라 다른 결과를 보였다. 모든 수종에서 시간이 지남에 따라 감소하는 경향을 보였으나 통계적인 차이는 없었다. 2016년 폭로 1년차 반응에서 구상나무와 소나무의 탄소고정효율이 감소하는 경향을 보였으나 (Figure 2a, Figure 2b) 졸참나무에서는 증가하는 경향을 보였다 (Figure 2c). 고온 1년차 처리에서 수종별 감소율은 구상나무는 약 36.6%, 소나무는 약 49.0%였다. 반면 졸참나무에서는 탄소고정효율이 약 5.5% 증가했다. 하지만 졸참나무의 탄소고정효율은 고온 2년차 처리에서 24.0%, 3년차 처리에서 20.9% 감소하는 경향을 보였다 (Figure 2c). 고온 2년차 처리에서 소나무는 1년차와 마찬가지로 29.0% 감소했다 (Figure 2b). 일반적으로 고온에 의한 환경스트레스는 주로 광합성계 단백질인 rubisco 활동을 저해하여 광합성 능력에 직·간접적으로 영향을 미치며 (Onoda et al., 2005), 고온 처리는 광합성에 영향을 주는 기공전도도와 증산속도를 감소시키기도 한다 (Saxe et al., 2001). 따라서 고온 처리에 대한 영향 정도는 수종에 따라 다르지만 장기간 지속될 경우 탄소고정효율 감소가 이어져 생장 촉진이 저하 될 수 있다.
3.3 고온과 고농도 CO2 처리에 따른 생리적 반응 특성
고온 및 고농도 CO2 변화에 따른 연도와 수종별 순광합성율 결과는 Figure 1에서 보는 바와 같다. 본 연구에서도 이전 연구와 유사하게 (Saxe et al., 2001; Ainsworth and Rogers, 2007) CO2 증가에 따른 광합성 속도 증가를 2016년 졸참나무, 2017년 구상나무에서 확인하였다. 소나무도 통계적 차이는 없었지만 CO2 시비효과로 인해 순광합성율이 증가하는 경향을 보였다 (Figure 1). 특히 2016년 졸참나무와 2017년 구상나무에서 뚜렷하게 증가하였으며 증가율은 각각 약 153.1%, 62.1%였다 (Figure 1c, Figure 1a).
광합성에서 rubisco의 활성과 함량을 반영하는 탄소고정효율의 경우 (Farquhar et al., 1980), 2016년 졸참나무를 제외한 구상나무와 소나무 모두 eC-eT에서 aC-aT 보다 감소하는 경향을 보였다 (Figure 2). 1년차 eC-eT 처리에서 수종별 감소율은 구상나무는 약 8.0%, 소나무는 약 22.7%였다 (Figure 2a, Figure 2b). 2년차 소나무는 14.8% 감소하는 경향을 보였으나 eC-eT 처리에서 CO2 시비효과를 통해 탄소고정효율 감소가 개선되는 경향을 보였다 (Figure 2b). 수종별 완화율은 구상나무는 약 45.1%, 소나무는 약 51.5%, 2년차에는 20.3% 였다. 졸참나무의 경우 2016년 이후 순광합성율과 탄소고정능력이 급격히 감소하기 시작했다 (Figure 2c). 2017년 eC-eT 처리구에서 고온처리에 비해 약 24.0% 감소하였다. 또한 해가 지남에 따라 2017년, 2018년 순광합성율이 약 35.7%, 9.9% 감소하였다 (Figure 1c, Figure 2c).
고온과 고농도 CO2 조건에 폭로한 기간에 따라 순광합성율과 탄소고정효율은 수종별, 연도별로 다르게 나타났다 (Figure 1, Figure 2). 기존 연구에서는 순광합성율과 탄소고정효율이 일반적으로 증가하는 것으로 파악되었으나 (Ainsworth and Rogers, 2007), 광합성이 저하되거나 차이가 발생하지 않은 경우도 보고되었다 (Ge et al., 2012). 이렇게 광합성 능력이 달라지는 요인으로는 수종별 적응기작의 변화와도 관련이 있겠으나, 질소가 제한된 환경에서는 잎 내 질소함량 (Hikosaka and Terashima, 1995)을 고려해야 한다. 잎 내 질소 함량 감소는 rubisco, 엽록소, 수용성 단백질 감소로 이어져 탄소고정능력이 저하될 수 있기 때문이다 (Tissue et al., 1999). Yin (2002)의 연구에서는 상대적으로 활엽수에서 잎의 질소 함량 감소 폭이 크다고 한다.
본 연구에서 실험기간 중 실험 화분 내 질소 시비를 하지 않았으므로, 3년간 eC-eT 처리에 의해 졸참나무의 광합성 능력이 저감된 것은 잎 내 질소 결핍과 관련 있을 수 있음을 고려해야한다. 따라서 수종별 탄소고정능력 변화 기작에 대한 정확한 이해를 위해서는 추가적인 잎의 양분 분석이 필요하다.
3.4 생장특성 (생물량 분배)
고온 및 고온·고농도 CO2 처리가 수종별 생장에 미치는 영향을 알아보기 위해 실시된 모든 개체의 부위별 생물량과 T/R은 Figure 3과 같다. 선행연구에 따르면, 고온에서 수목의 생장은 수종에 따라 증가 (Danby and Hik, 2007), 감소 (Valerie A et al., 2000) 혹은 변화가 없는 (Volder et al., 2007)등 반응이 다양하다. 본 연구에서 모든 처리에 대한 소나무와 졸참나무의 양분, 수분, 환경 조건은 동일하게 진행하였다. 소나무의 지상부, 지하부, 전체 생물량은 처리구에 따라 명확한 반응 차이를 보였으나 (Figure 3a, Figure 3c), 졸참나무는 지하부에서만 통계적인 차이를 보였다 (Figure 3b).
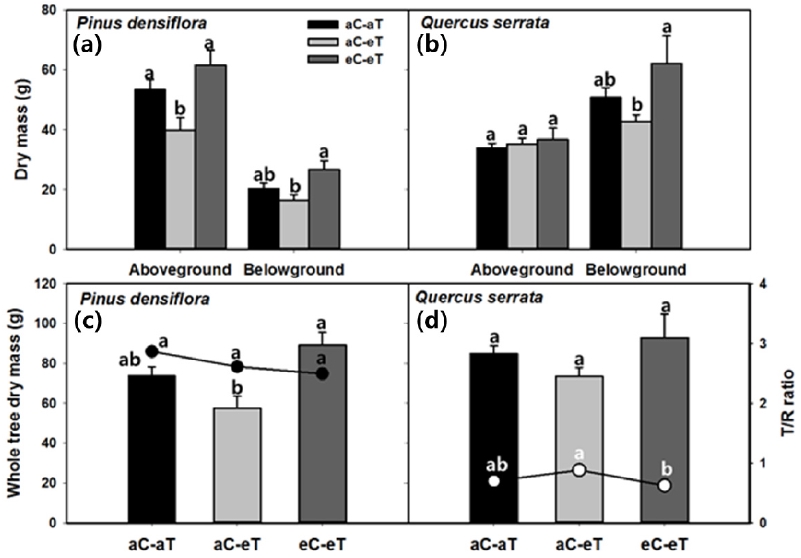
Mean ± SE of (a), (c) P. densiflora and (b), (d) Q. serrata dry mass for each treatment: ambient (aC-aT), ambient CO2 & warmed treatment (aC-eT), elevated CO2 & warmed treatment (eC-eT) measured at the time of harvest in 2018. (a), (b) Aboveground and Belowground biomass. (c), (d) Total tree biomass and T/R (Aboveground/ Belowground) ratio. Different letters within a subpanel represent statistical differences (p<.05) based on the Duncan test. Error bar indicates standard error.
aC-eT 처리구에서 소나무와 졸참나무 모두 생물량이 감소하는 경향을 보였다 (Figure 3). 졸참나무는 지하부 생산량이 42.7 ± 2.3 g으로 유의하게 낮았지만 지상부와 총 생물량은 처리에 따라 통계적인 차이를 보이지 않았다 (Figure 3b, Figure 3c). 그러나 소나무에서는 지상부, 지하부, 총 생물량이 각각 39.9 ± 4.2 g, 16.4 ± 2.1 g, 57.5 ± 6.1 g로 유의하게 낮았다 (Figure 3a, Figure 3b). Ainsworth and Rogers (2007)가 일반적으로 CO2 시비효과에 의한 광합성 촉진은 식물의 생장에 직접적인 영향을 미쳐 생물량이 증가한다고 보고한 것과 유사하게, 고농도의 CO2가 폭로되는 eC-eT 처리구에서 두 수종의 부위별 생물량이 증가하였다. 졸참나무는 지하부만 62.2 ± 9.2 g으로 유의하게 높았던 반면, 소나무는 지상부, 지하부, 총 생물량이 각각 61.7 ± 4.9 g, 26.8 ± 2.8 g, 89.1 ± 6.5 g로 유의하게 증가하였다. 이러한 생장 반응은 수종에 따라 양분유효도, 온도, 수분 이용 등과 같은 환경 변수에 의해 복합적으로 영향을 받기 때문에 (Oren et al., 2001; Norby et al., 2005; McCarthy et al., 2010) 다양한 결과를 보일 수 있다.
T/R은 대표적인 환경 스트레스의 지표로서 수분과 양분 제한 조건에서 식물체는 뿌리 활동을 증진시키기 위해 뿌리의 생물량을 늘려 T/R이 낮아진다 (Valerie A et al., 2000). 한편 대기 중 CO2 농도 증가 조건에서는 수목의 T/R이 감소하거나 증가하는 등 다양한 반응을 보인다 (Way and Oren, 2010). 본 연구에서 수종별 T/R을 살펴본 결과, 소나무는 대조구에서 가장 높았으며 고온처리와 고온·CO2 처리에서 감소하는 경향을 보였다 (Figure 3c). 선행 연구에서도 aC-eT 처리 환경에서 소나무와, 가문비나무의 T/R이 감소하였는데 이는 수분 스트레스와 관련이 있는 것으로 보고되었다 (Yin et al., 2008). 반면 졸참나무의 경우, aC-eT 처리구에서 뚜렷하게 높은 값을 보였다 (Figure 3d). 일반적으로 대기 중 온도 상승은 지상부를 약 40% 증가시키고, 지하부를 약 30% 감소시킨다는 기존연구에 비추어 볼 때 (Way and Oren, 2010), 졸참나무는 고온처리로 인해 지상부의 생장이 지하부 보다 더 증가한 것으로 판단된다.
전체 생물량에 대한 부위별 비율 (잎, 줄기·가지, 뿌리)은 소나무에서는 선행 연구 (Quentin et al., 2015)에서와 같이 모두 처리구에 따라 뚜렷한 차이를 보이지 않았다 (Figure 4a). 반면 졸참나무에서는 aC-eT에서 뿌리 비율이 유의하게 감소하였다 (Figure 4b). Way and Oren (2010)는 고온처리 환경에서 자란 수목은 뿌리보다 잎에 더 많은 탄소를 분배한다는 결론을 내렸다. 그러나 소나무는 지상부가 많은 비율을, 졸참나무는 지하부가 많은 비율을 차지하여 두 수종의 분배 양상이 다르게 나타났다. 모든 처리구에 대해 부위별 비율이 크게 변하지 않은 소나무와 달리 졸참나무는 aC-eT에서 지하부가 약 18.3% 감소하고, eC-eT에서 CO2에 의한 완화효과로 약 14.3% 증가하며 회복하는 경향을 보였다 (Figure 4). 이렇게 수종에 따라 부위별 반응 차이가 나는 이유는 환경적, 유전적 조건이 뿌리의 수분 섭취와 잎에서의 수분 손실 사이의 균형 조절에 영향을 주는 것으로 생각된다.
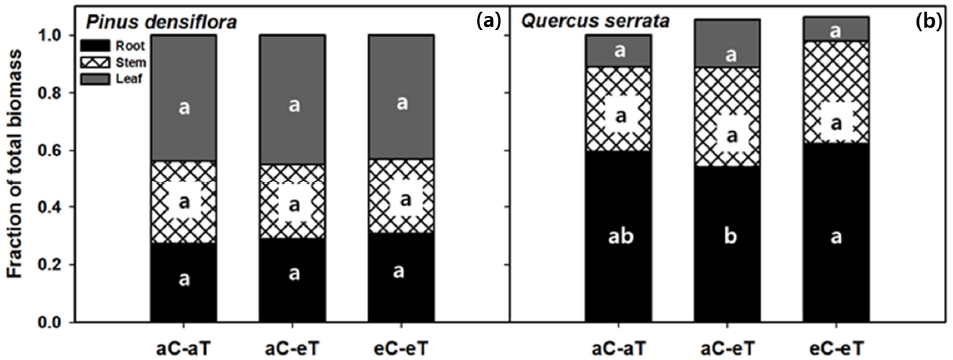
Fraction of the total biomass shown for three tree components (Root, black; Stem, hatched; Leaf, grey) in each treatments. Treatment combinations are: ambient (aC-aT), ambient CO2 & warmed treatment (aC-eT), elevated CO2 & warmed treatment (eC-eT). Different letters within a subpanel represent statistical differences (p<.05) based on the Duncan test. (a) P. densiflora and (b) Q. serrata
4. 결론
본 연구는 대기 중 온도와 CO2 농도가 인위적으로 조절되는 기후변화 대응 온실인 TGC와 CTGC를 이용하여 미래 기후변화 환경에서 2년생 구상나무, 소나무, 졸참나무의 생장 및 생리적 반응 특성을 3년간 조사하였다. 광합성과 탄소고정반응은 수종과 폭로 기간에 따라 다양한 반응을 보였다. 침엽수인 구상나무와 소나무의 광합성 특성은 고온처리에서 감소하였지만 CO2 시비를 통해 완화되는 효과가 있었다. 반면 활엽수인 졸참나무는 증가된 순광합성율과 탄소고정능력이 처리 2년차부터 뚜렷하게 감소하였고 CO2 시비효과가 미미했다. 대기 중 온도와 CO2 증가 하에서 3년간 처리된 수종별 생물량에 대해서 전체와 지상부, 지하부의 뚜렷한 차이는 나타나지 않았다. 그러나 소나무와 졸참나무에서 지상부와 지하부의 비 (T/R)는 서로 상반된 반응을 보였다. 소나무의 T/R은 대조구, aC-eT, eC-eT 처리구 순서대로 감소하는 경향을 보였다. 반면 졸참나무는 고온에서 지상부 생장이 지하부 보다 더 증가하여 T/R이 증가하였다. 하지만 eC-eT에서 다시 두 수종의 전체 생물량이 증가하였는데 이는 CO2의 시비효과인 것으로 판단된다. 결과적으로 기온과 CO2 변화에 따른 생장 혹은 생리적 반응은 수종과 처리 기간에 따라 차이를 나타냈다. 또한 고온 및 고농도 CO2 처리를 지속하여 조사한 결과, 처리 기간에 따라 반응이 통계적으로 의미 있는 차이를 나타냈다는 것에 본 연구결과의 의의가 있지만, 본 연구에서는 묘목을 이용하여 실험을 진행하였으므로 장기간 생장단계별 반응을 지켜 볼 필요가 있다. 아울러 광합성 능력과 밀접한 관련이 있는 잎의 양분에 대한 추가적인 분석과 단기간이 아닌 장기적인 관찰이 요구된다.
References
-
Ainsworth EA, Rogers A. 2007. The response of photosynthesis and stomatal conductance to rising [CO2]: mechanisms and environmental interactions. Plant, Cell Environment 30: 258-270.
[https://doi.org/10.1111/j.1365-3040.2007.01641.x]
-
Bereiter B, Eggleston S, Schmitt J, Nehrbass‐Ahles C, Stocker TF, Fischer H, Kipfstuhl S, Chappellaz J. 2015. Revision of the EPICA Dome C CO2 record from 800 to 600 kyr before present. Geophysical Research Letters: 542-549.
[https://doi.org/10.1002/2014GL061957]
-
Chun JH, Lee CB. 2013. Assessing the Effects of Climate Change on the Geographic Distribution of Pinus densiflora in Korea using Ecological Niche Model. Korean Journal of Agricultural and Forest Meteorology 15: 219-233. (In Korean with English abstract).
[https://doi.org/10.5532/KJAFM.2013.15.4.219]
-
Danby RK, Hik DS. 2007. Responses of white spruce (Picea glauca) to experimental warming at a subarctic alpine treeline. Global Change Biology 13: 437-451.
[https://doi.org/10.1111/j.1365-2486.2006.01302.x]
-
Farquhar GD, S. von Caemmerer, J. A Berry. 1980. A biochemical model of photosynthetic CO2 assimilation in leaves of C3 species. Planta 149: 78-90.
[https://doi.org/10.1007/BF00386231]
-
Ge Z, Zhou X, Kellomäki S, Peltola H, Martikainen P, Wang K. 2012. Acclimation of photosynthesis in a boreal grass (Phalaris arundinacea L.) under different temperature, CO2, and soil water regimes. Photosynthetica 50: 141-151.
[https://doi.org/10.1007/s11099-012-0014-x]
-
Hikosaka K, Terashima I. 1995. A model of the acclimation of photosynthesis in the leaves of C3 plants to sun and shade with respect to nitrogen use. Plant, Cell Environment 18: 605-618.
[https://doi.org/10.1111/j.1365-3040.1995.tb00562.x]
-
Huang J-G, Bergeron Y, Denneler B, Berninger F, Tardif J. 2007. Response of forest trees to increased atmospheric CO2. Critical Reviews in Plant Sciences 26: 265-283.
[https://doi.org/10.1080/07352680701626978]
- IPCC. 2013. Climate change 2013: The physical science basis. In. Cambridge University Press, Cambridge.
- IPCC. 2018. Global warming of 1.5°C An IPCC Special Report on the impacts of global warming of 1.5°C above pre-industrial levels and related global greenhouse gas emission pathways, in the context of strengthening the global response to the threat of climate change, sustainable development, and efforts to eradicate poverty. In. Cambridge University Press, Cambridge.
- KFRI. 2014a. Growth and Physiological Response Characteristics of Major Species according to Light Intensity and Soil Moisture Environment: Korea Forest Research Institute. (In Korean)
- KFRI. 2014b. Predicting the Changes of Productive Areas for Major Tree Species under Climate Change in Korea. In. p 240. (In Korean)
- Kim PG, Lee EJ. 2001. Ecophysiology of Photosynthesis 1: Effects of Light Intensity and Intercellular CO2 Pressure on Photosynthesis. Korean Journal of Agricultural and Forest Meteorology 3:126-133. (In Korean with English abstract).
- Koo KA, Park WK, Kong WS. 2001. Dendrochronological analysis of Abies koreana W. at Mt. Halla, Korea: effects of climate change on the growths. The Korean Journal of Ecology 24:281-288. (In Korean with English abstract).
-
Leakey AD, Ainsworth EA, Bernacchi CJ, Rogers A, Long SP, Ort DR. 2009. Elevated CO2 effects on plant carbon, nitrogen, and water relations: six important lessons from FACE. Journal of Experimental Botany 60: 2859-2876.
[https://doi.org/10.1093/jxb/erp096]
-
Lee JS, Usami T, Oikawa T. 2001. High performance of CO2–temperature gradient chamber newly built for studying the global warming effect on a plant population. Ecological research 16: 347-358.
[https://doi.org/10.1046/j.1440-1703.2001.00398.x]
- Lim JH, Woo SY, Kwon MJ, Chun JH, Shin MH. 2006. Photosynthetic capacity and water use efficiency under different temperature regimes on healthy and declining Korean fir in Mt. Halla. Journal of Korean Forestry Society 95: 705-710. (In Korean with English abstract).
-
Lüthi D, Le Floch M, Bereiter B, Blunier T, Barnola J-M, Siegenthaler U, Raynaud D, Jouzel J, Fischer H, Kawamura K. 2008. High-resolution carbon dioxide concentration record 650,000-800,000 years before present. Nature 453: 379.
[https://doi.org/10.1038/nature06949]
-
Marcott SA, Shakun JD, Clark PU, Mix AC. 2013. A reconstruction of regional and global temperature for the past 11,300 years. Science 339: 1198-1201.
[https://doi.org/10.1126/science.1228026]
-
McCarthy HR, Oren R, Johnsen KH, Gallet-Budynek A, Pritchard SG, Cook CW, LaDeau SL, Jackson RB, Finzi AC. 2010. Re-assessment of plant carbon dynamics at the Duke free-air CO2 enrichment site: interactions of atmospheric [CO2] with nitrogen and water availability over stand development. New Phytologist 185: 514-528.
[https://doi.org/10.1111/j.1469-8137.2009.03078.x]
-
Medlyn B, Badeck FW, De Pury D, Barton C, Broadmeadow M, Ceulemans R, De Angelis P, Forstreuter M, Jach M, Kellomäki S. 1999. Effects of elevated [CO2] on photosynthesis in European forest species: a meta‐analysis of model parameters. Plant, Cell Environment 22: 1475-1495.
[https://doi.org/10.1046/j.1365-3040.1999.00523.x]
-
Norby RJ, DeLucia EH, Gielen B, Calfapietra C, Giardina CP, King JS, Ledford J, McCarthy HR, Moore DJP, Ceulemans R, De Angelis P, Finzi AC, Karnosky DF, Kubiske ME, Lukac M, Pregitzer KS, Scarascia-Mugnozza GE, Schlesinger WH, Oren R. 2005. Forest response to elevated CO2 is conserved across a broad range of productivity. Proceedings of the National Academy of Sciences of the United States of America 102: 18052-18056.
[https://doi.org/10.1073/pnas.0509478102]
-
Norby RJ, Warren JM, Iversen CM, Medlyn B, McMurtrie RE. 2010. CO2 enhancement of forest productivity constrained by limited nitrogen availability. Proceedings of the National Academy of Sciences of the United States of America 107: 19368-19373.
[https://doi.org/10.1073/pnas.1006463107]
-
Onoda Y, Hikosaka K, Hirose T. 2005. The balance between RuBP carboxylation and RuBP regeneration: a mechanism underlying the interspecific variation in acclimation of photosynthesis to seasonal change in temperature. Functional Plant Biology 32: 903-910.
[https://doi.org/10.1071/FP05024]
-
Oren R, Ellsworth DS, Johnsen KH, Phillips N, Ewers BE, Maier C, Schäfer KV, McCarthy H, Hendrey G, McNulty SG. 2001. Soil fertility limits carbon sequestration by forest ecosystems in a CO2-enriched atmosphere. Nature New Biology 411: 469.
[https://doi.org/10.1038/35078064]
-
Quentin AG, Crous KY, Barton CVM, Ellsworth DS. 2015. Photosynthetic enhancement by elevated CO2 depends on seasonal temperatures for warmed and non-warmed Eucalyptus globulus trees. Tree Physiology 35: 1249-1263.
[https://doi.org/10.1093/treephys/tpv110]
-
Sage RF, Sharkey TD, Seemann JR. 1989. Acclimation of photosynthesis to elevated CO2 in five C3 species. Plant Physiology 89: 590-596.
[https://doi.org/10.1104/pp.89.2.590]
-
Saxe H, Cannell MG, Johnsen Ø, Ryan MG, Vourlitis G. 2001. Tree and forest functioning in response to global warming. New Phytologist 149: 369-399.
[https://doi.org/10.1046/j.1469-8137.2001.00057.x]
-
Sendall KM, Reich PB, Zhao C, Jihua H, Wei X, Stefanski A, Rice K, Rich RL, Montgomery RA. 2015. Acclimation of photosynthetic temperature optima of temperate and boreal tree species in response to experimental forest warming. Global Change Biology 21: 1342-1357.
[https://doi.org/10.1111/gcb.12781]
-
Seo DJ, Kim HC, Lee HS, Lee SJ, Lee WY, Han SH, Kang JW. 2016. Review of Long-term Climate Change Research Facilities for Forests. Korean Journal of Agricultural Forest Meteorology 18: 274-286. (In Korean with English abstract).
[https://doi.org/10.5532/KJAFM.2016.18.4.274]
-
Shin MY, Lee DK. 2008. Long‐term prediction of forest sustainability of the natural deciduous forest in Pyungchang area of Gangwon province, Korea. Forest Science Technology 4: 28-34. (In Korean with English abstract).
[https://doi.org/10.1080/21580103.2008.9656334]
-
Stirling C, Heddell-Cowie M, Jones M, Ashenden T, Sparks T. 1998. Effects of elevated CO2 and temperature on growth and allometry of five native fast-growing annual species. New Phytologist 140: 343-354.
[https://doi.org/10.1046/j.1469-8137.1998.00273.x]
-
Tissue DT, Griffin KL, Ball JT. 1999. Photosynthetic adjustment in field-grown ponderosa pine trees after six years of exposure to elevated CO2. Tree Physiology 19: 221-228.
[https://doi.org/10.1093/treephys/19.4-5.221]
- Valerie A B, Glenn Patrick J, Bruce P F. 2000. Reduced growth of Alaskan white spruce in the twentieth century from temperature-induced drought stress. Nature
-
Volder A, Gifford RM, Evans JR. 2007. Effects of elevated atmospheric CO2, cutting frequency, and differential day/night atmospheric warming on root growth and turnover of Phalaris swards. Global Change Biology 13: 1040-1052.
[https://doi.org/10.1111/j.1365-2486.2007.01321.x]
-
Way DA, Oren R. 2010. Differential responses to changes in growth temperature between trees from different functional groups and biomes: a review and synthesis of data. Tree Physiology 30: 669-688.
[https://doi.org/10.1093/treephys/tpq015]
- Woodward FI. 1987. Climate and plant distribution: Cambridge University Press.
-
Yin HJ, Liu Q, Lai T. 2008. Warming effects on growth and physiology in the seedlings of the two conifers Picea asperata and Abies faxoniana under two contrasting light conditions. Ecological Research 23: 459-469.
[https://doi.org/10.1007/s11284-007-0404-x]
-
Zhu P, Zhuang Q, Ciais P, Welp L, Li W, Xin Q. 2017. Elevated atmospheric CO2 negatively impacts photosynthesis through radiative forcing and physiology-mediated climate feedback. Geophysical Research Letters 44: 1956-1963.
[https://doi.org/10.1002/2016GL071733]